1.1 Introduction
The study of all aspects of energy and energy transformations, including power generation, refrigeration, and relationships among the properties of matter.
One of the most fundamental laws of nature is the conservation of energy principle, which states that during an interaction, energy can change from one form to another but the total amount of energy remains constant. In other words, neither energy can be destroyed nor created.
We can't calculate the exact value of the system's energy (defined by the observer) or universe. Still, we can determine changes in the energy content ( Δ E) of the body or any other system, which is equal to the difference between the energy input and the energy output.
The energy balance equation can be written for the system as :
Zeroth law :
If Body A and B are at thermal equilibrium and Body A and C are at thermal equilibrium, then B and C will also be in thermal equilibrium.
First law :
The first law of thermodynamics is simply an expression of the conservation of energy principle, and it asserts that energy is a thermodynamic property. Due to the limitation of the first law of thermodynamics, the second law of thermodynamics was proposed as the first law cannot predict the direction of a reaction based on spontaneity. We will discuss the first law of thermodynamics case-wise in detail in the upcoming unit.
Second law :
It tells about the feasibility of the reaction. It asserts that energy has quality as well as quantity, and actual processes occur in the direction of decreasing the quality of energy.
For instance, a cup of hot coffee left on a table eventually cools, but a cup of cool coffee in the same room never gets hot by itself once it is transferred to the surroundings i.e. heat flow in the direction of decreasing temperature.
Third law :
The entropy of the pure crystalline substance is zero at absolute zero kelvin temperature.
1.2 System, Surrounding and Boundary
A system is defined as a quantity of matter or a region in space chosen for study.
While the mass or region outside the system is called the surroundings.
The real or imaginary surface that separates the system from its surroundings is called the boundary. The boundary of a system can be fixed or movable. The boundary is the contact surface shared by both the system and the surroundings.
Open, Closed, and Isolated System :
Systems may be considered to be closed or open, depending on whether a fixed mass or a fixed volume in space is chosen for study.
A closed system (or control mass) consists of a fixed amount of mass, and no mass can cross its boundary but energy in the form of heat or work, can cross the boundary.
Even if energy is not allowed to cross the boundary, the system is called an isolated system.
An open system (or control volume), is a properly selected region in space. Both mass and energy can cross the boundary of a control volume.
Application of Open System in Control Flow Device:
For open system, usually encloses a device that involves mass flow such as a compressor, turbine, water heater, car radiator, or nozzle. Flow through these devices is best studied by selecting the region within the device as the control volume instead of the control mass.
The boundaries of a control volume are called a control surface, and they can be real or imaginary.
A control volume can be fixed in size and shape or it may involve a moving boundary. A control volume can also involve heat and work interactions just as a closed system, in addition to mass interaction.
1.2.1 Nozzle
To analyze the flow of air through a nozzle, a good choice for the control volume would be the region within the nozzle. The inner surface of the nozzle forms the real part of the boundary, and the entrance and exit areas form the imaginary part. It is fixed in size and shape.
1.2.2 Water Heater
We can concentrate our attention on the volume formed by the interior surfaces of the tank and consider the hot and cold water streams as mass leaving and entering the control volume. The interior surfaces of the tank form the control surface for this case, and mass crosses the control surface at two locations.
1.3 Properties of a system
Any characteristic of a system is called a property like mass, volume, temperature, and pressure. Properties are considered to be either intensive or extensive.
Intensive properties are those that are independent of the mass of a system, such as temperature, pressure, and density. Extensive properties are those whose values depend on the size—or extent—of the system. Total mass, total volume, and total momentum are some examples of extensive properties.
Extensive properties per unit mass are called specific properties. For example- specific volume (v =V/m) and specific total energy (e = E/m).
1.4 Density and Specific Gravity
Density is defined as mass per unit volume. The reciprocal of density is the specific volume v, which is defined as volume per unit mass.
The density of a substance, in general, depends on temperature and pressure. The density of most gases is proportional to pressure and inversely proportional to temperature. As liquids and solids are essentially incompressible substances, the variation of their density with pressure is negligible.
The density of liquids and solids depends more strongly on temperature than pressure.
Specific gravity or Relative density can be defined as the ratio of the density of a substance to the density of some standard substance at a specified temperature (usually water at 4°C, for which density of water =1000 kg/m3).
For example: The density of mercury with a specific gravity of 13.6, will be 13600 kg per cubic metre.
Specific weight (Ys)
The weight of a unit volume of a substance is called a specific weight.
1.5 State and Equilibrium
The word equilibrium implies a state of balance. In an equilibrium state, there are no unbalanced potentials (or driving forces) within the system. A system in equilibrium experiences no changes when it is isolated from its surroundings.
A system is not in thermodynamic equilibrium unless the conditions of all the relevant types of equilibrium are satisfied given below :
- Thermal Equilibrium- a system is in thermal equilibrium if the temperature is the same throughout the entire system and heat flow as a driving force is absent.
- Mechanical Equilibrium (related to pressure) - a system is in mechanical equilibrium if there is no change in pressure at any point of the system with time. However, the pressure may vary within the system with elevation as a result of gravitational effects but the variation of pressure as a result of gravity in most thermodynamic systems is relatively small and usually disregarded.
- Phase Equilibrium - If a system involves two phases, it is in phase equilibrium when the mass of each phase reaches an equilibrium level and stays there.
- Chemical Equilibrium- a system is in chemical equilibrium if its chemical composition does not change with time and no chemical reactions occur.
The State Postulate
The state of a system is described by its properties. Once a sufficient number of properties are specified, the rest of the properties assume certain values automatically.
The number of properties required to fix the state of a system is given by the state postulate: The state of a simple compressible system is completely specified by two independent, intensive properties.
The state postulate requires that the two properties specified be independent to fix the state. Two properties are independent if one property can be varied while the other one is held constant.
Temperature and specific volume are always independent properties, and together they can fix the state of a simple compressible system. Temperature and pressure are independent properties for single-phase systems but are dependent properties for multiphase systems.
For example- During a phase-change process between liquid and vapor, temperature and pressure are not sufficient to fix the state of a two-phase system.
Based on this concept, we will discuss the Gibbs-phase rule in an upcoming unit.
1.6 Process and Cycle
Any change that a system undergoes from one equilibrium state to another is called a process.
The series of states through which a system passes during a process is called the path of the process.
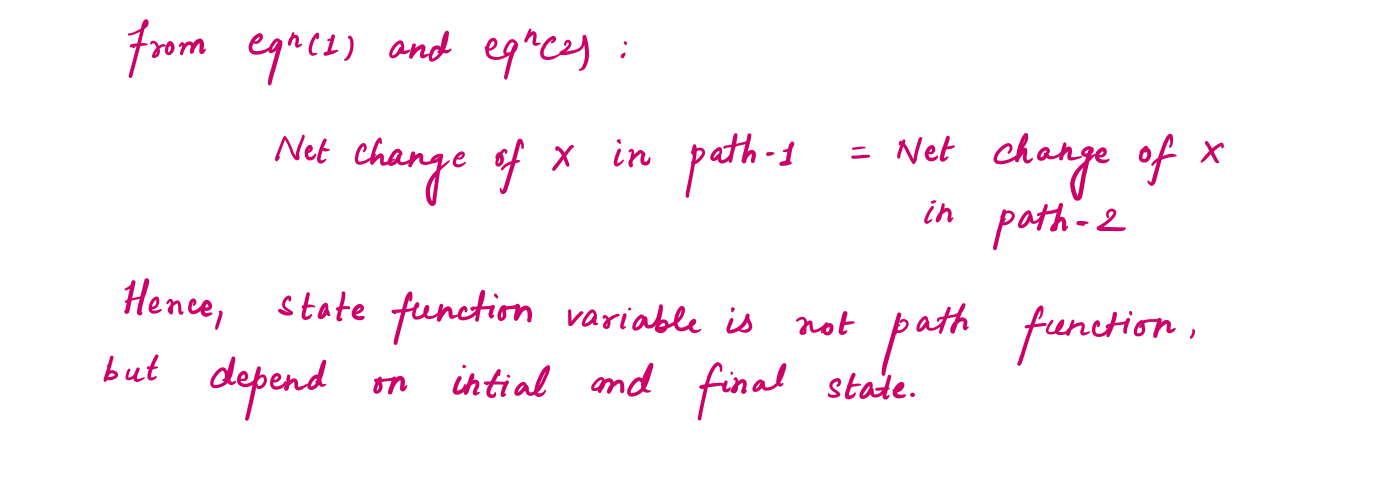
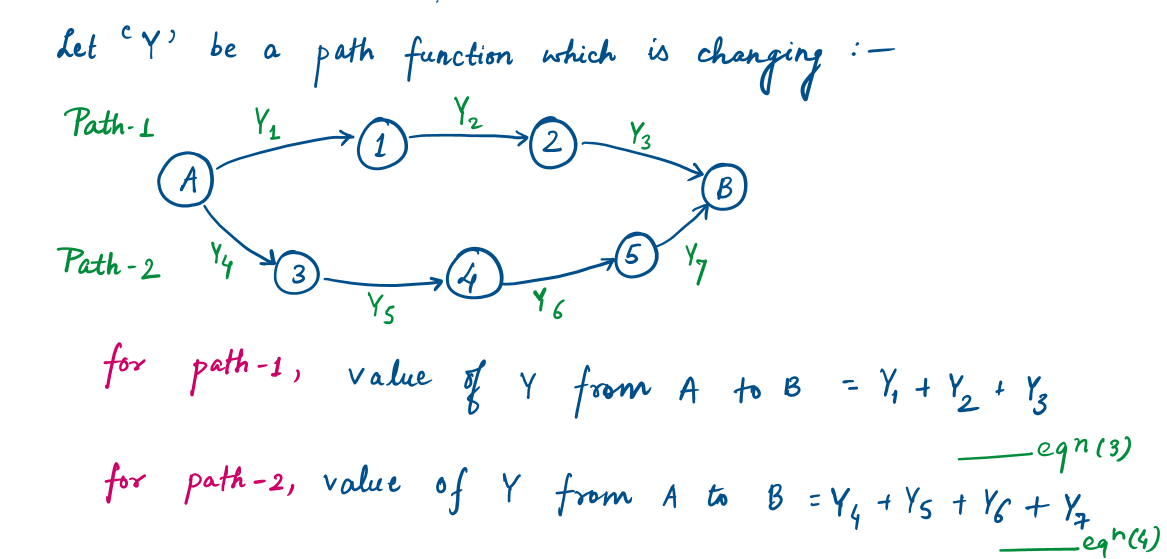
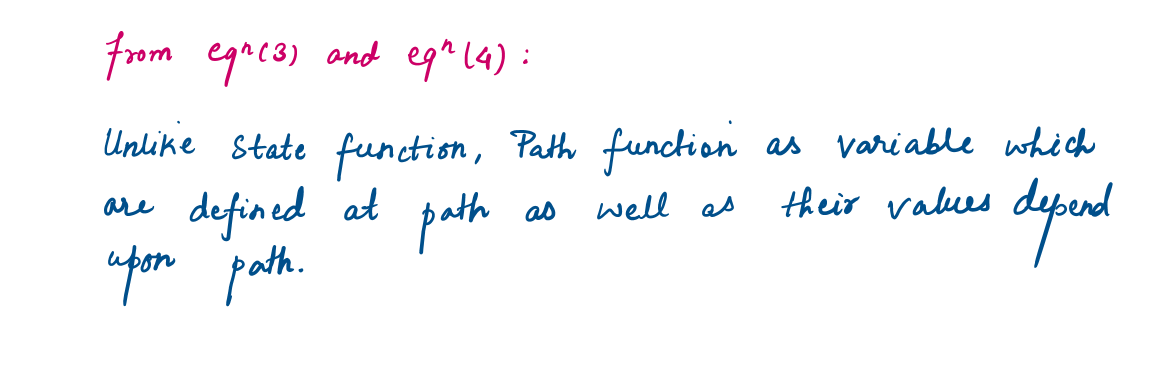
Quasi-equilibrium process
When a process proceeds in such a manner that the system remains infinitesimally close to an equilibrium state at all times, it is called a quasistatic, or quasi-equilibrium, process.
A quasi-equilibrium process is a sufficiently slow process that allows the system to adjust itself internally so that properties in one part of the system do not change any faster than those at other parts.
For a better understanding, let's take an instance with two contrasting case
Case 1: Non-Quasi-Equilibrium State (Sudden and Irreversible Process)
When a gas in a piston-cylinder device is compressed suddenly, the molecules near the face of the piston will not have enough time to escape and they will have to pile up in front of the piston, thus creating a high-pressure region there. Because of this pressure difference, the system can no longer be said to be in equilibrium, and this makes the entire process non-quasi-equilibrium.
Case 2: Quasi-Equilibrium State (Slow and Reversible Process)
If the piston is moved slowly, the molecules will have sufficient time to redistribute and there will not be a molecule pileup in front of the piston. As a result, the pressure inside the cylinder will always be nearly uniform and will rise at the same rate at all locations. Since equilibrium is maintained at all times, this is a quasi-equilibrium process.
Reason for using Quasi-static model for actual process with negligible error
- they are easy to analyze
- work-producing devices deliver the most work when they operate on quasi-equilibrium processes.
Isobaric, Isochoric, and Isothermal process
An isothermal process is a process during which the temperature T remains constant; an isobaric process is a process during which the pressure P remains constant; and an isochoric (or isometric) process is a process during which the specific volume v remains constant.
A Cyclic Process
A system is said to have undergone a cycle if it returns to its initial state at the end of the process. For a cycle the initial and final states are identical.
1.6.1 The Steady-Flow Process
The steady-flow process can be defined as a process during which a fluid flows through a control volume steadily which means no change with time.
What is the difference between the terms uniform and steady in thermodynamics?
The term steady implies no change with time. The opposite of steady is unsteady, or transient. The term uniform implies no change in location over a specified region. The fluid properties can change from point to point within the control volume, but at any fixed point they remain the same during the entire process.
Therefore, the volume V, the mass m, and the total energy content E of the control volume remain constant during a steady flow process.
Steady-flow conditions can be closely approximated by devices that are intended for continuous operation such as turbines, pumps, boilers, condensers, heat exchangers power plants, or refrigeration systems.
Some cyclic devices, such as reciprocating engines or compressors, do not satisfy any of the conditions stated above because the flow at the inlets and the exits will be pulsating and not steady; the fluid properties periodically vary with time and the flow through these devices can still be analyzed as a steady-flow process by using time-averaged values for the properties
1.7 The Concept of Continuum
Matter is made up of tiny atoms, which are spread out in gases. However, instead of thinking about individual atoms, we often treat matter as one smooth, continuous thing, called the continuum. This makes it easier to study because we assume the properties of the substance (like density) change smoothly, without any sudden jumps. This idea works well as long as the space between atoms is very small compared to the size of the system.
For example, in normal conditions, oxygen molecules are very small and don’t travel far before bumping into each other. But in places with very low pressure, like high up in the atmosphere, we have to think about individual molecules because the space between them becomes much larger.